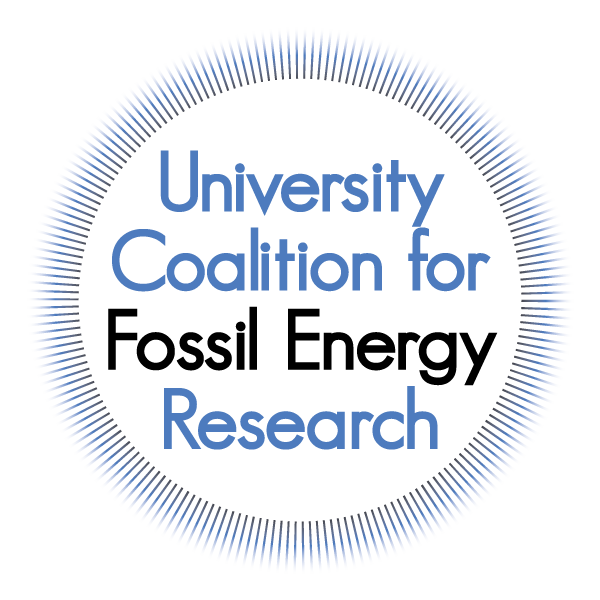
Two additional grants have been awarded to Texas A&M University in the U.S. Department of Energy (DOE) National Energy Technology Laboratory (NETL) University Coalition for Fossil Energy Research (UCFER) program, bringing the total to four over the six-year, $20 million project.
Texas A&M is one of UCFER’s Founding Member Universities, and the UCFER program is overseen at Texas A&M University by the Texas A&M Energy Institute. The goals of the project are to identify, select, execute, review and disseminate knowledge from research that will improve the efficiency of production and use of fossil energy resources while minimizing the environmental impacts and reducing greenhouse gas emissions.
In UCFER’s Second Round of Solicitations (2016), two Texas A&M proposals were selected for awards. A total of 25 proposals were received and reviewed by the Technical Advisory Council, Core Competency Board, external reviewers, and the Executive Council. Total funding available for this round of proposals was $2.08 million and six projects were approved for funding.
- Dr. Eric Petersen (PI), Nelson-Jackson Professor; and Dr. Waruna Kulatilaka (co-PI), associate professor; both in the Department of Mechanical Engineering and Texas A&M Energy Institute faculty affiliates, led the first project, “Validation of CFD Models for Turbulent, Supercritical CO2 Combustion,” which was funded for $398,832.
- Dr. Benjamin Wilhite (PI), associate professor in the Department of Chemical Engineering and a Texas A&M Energy Institute faculty affiliate; and Dr. Jaime Grunlan (co-PI), Linda & Ralph Schmidt ’68 Professor in the mechanical engineering department and a Texas A&M Energy Institute faculty affiliate; led the second project, “Layer-by-Layer Functional Thin Film Coatings for Enhanced Light Gas Separations,” which was funded for $236,839.
Most recently, in UCFER’s Third Round of Solicitations (2018), two additional Texas A&M proposals were selected for awards. A total of 81 proposals were received and reviewed by the Technical Advisory Council, Core Competency Board, external reviewers, other coalition faculty, and the Executive Council. Total funding available for this round of proposals was $4.099 million and 11 projects were approved for funding.
- Dr. Richard Miles (PI), a professor of aerospace engineering; along with Dr. Albina Tropina (Co-PI), a research professor in the Department of Aerospace Engineering, and Dr. Christopher Limbach (Co-PI), an assistant professor in the Department of Aerospace Engineering; led the first project, “Seed-Free MHD Topping Cycle for Coal and Gas Fired Power Generation,” which was funded for $424,100.
- Dr. Hongcai (Joe) Zhou (PI), a professor of chemistry and a Texas A&M Energy Institute faculty affiliate, led the second project, “Porous Polymer Network Membranes and Porous Molecular Additive for Post Combustion CO2 Capture,” which was funded for $203,000.
Seed-Free MHD Topping Cycle for Coal and Gas Fired Power Generation
The United States relies heavily on hydrocarbon energy sources for the generation of electrical power. These include petroleum, coal, syngas and natural gas. In 2016, about 30 percent of electric power in the US was generated by coal and 34 percent by natural gas. The conversion to electrical power for those hydrocarbons involves a combustion process of producing a high temperature which drives a power generating thermodynamic cycle. Many factors affect the efficiency of the thermodynamic cycle, but fundamentally it is determined by the temperature difference between the high temperature produced from combustion and the relatively cool temperature of the environment. Materials that are required for the operation of conventional power plants limit the peak operational temperature to much lower than the highest temperatures available from the combustion, leading to a significant reduction in the operational efficiency of conventional electrical generation processes.
Those materials limitations can be avoided by expanding the high temperature, combustion product laden gas through a nozzle to achieve high velocity in a wind tunnel and extracting electrical power directly from that high velocity gas by separating positive and negative charges as they pass through a magnetic field, leading to current flow through an external load. This process is called magnetohydrodynamic (MHD) power generation. After losing some of its energy to the MHD power extraction, the gas is still hot, and it can be subsequently used to heat a conventional power generator. Thus, the MHD process is termed a “topping cycle” and adds to the power generated by the conventional “bottoming cycle” which increases the overall efficiency of the process and reduces the amount of hydrocarbon fuel needed to service the electrical demand.
For the MHD process to be successful, the combustion product gas must conduct electricity. To do so the gas must contain a significant concentration of electrons and ions. Conductivity only occurs when neutral molecules are heated to high enough temperatures to ionize. The hot combusting gases do not have enough conductivity to achieve significant power extraction, even from very hot combustion with pure oxygen. This means that some method for augmenting the conductivity is required. In “conventional” MHD, that is achieved by adding up to 5 percent of an easily ionized species like potassium. That requires both the addition and subsequent removal of the seed material, which adds great complexity and cost to the MHD operation. As the temperature of the flow cools, the conductivity drops, so very high temperatures also need to be sustained through the MHD power extraction channel to maintain conductivity.
This research partnership between Texas A&M University and Princeton University seeks to develop an MHD “topping cycle” that does not rely on seeding the combustion products with an easily ionized species. Sustaining conductivity by external methods has the advantage of removing the seeding requirement and potentially extending the operability of MHD to lower temperatures and higher flow speeds since the conductivity is no longer directly related to thermal ionization. The research will be conducted in close collaboration with NETL personnel and will examine the possibility of using high voltage, short duration pulses to ionize the gas. The pulses only last for a few nanoseconds, have high enough voltage to achieve efficient ionization, and are applied through dielectric barrier walls at repetition rates high enough to maintain effective average conductivity. The discharges are guided by the magnetic field, so ionization is confined to the core of the flow, minimizing wall losses.
The power required to create the conductivity must be a fraction of the power extracted from the MHD process to be viable. Both computational modeling and experiments are directed toward understanding the fundamental physics underlying externally sustained conductivity and optimizing a topping cycle concept to mesh successfully with existing power generation facilities. The research is being conducted under the direction of Dr. Richard Miles.
Porous Polymer Network Membranes and Porous Molecular Additive for Post Combustion CO2 Capture
A major component of the electricity generated in the United States comes from the combustion of such fossil fuels as coal, oil and natural gas. Fossil fuel combustion produces a flue gas mixture of carbon dioxide, nitrogen, oxygen and trace corrosive pollutants. The separation and sequestration of carbon dioxide (CO2) from the other gases in effluent streams is of interest to industry and the greater public in the pursuit of cleaner energy production. A variety of physical and chemical processes for the separation of CO2 from flue gas has been extensively studied. One process of interest employs selective membrane filters to isolate CO2 from other gases. A membrane filter system is attractive due to its low energy consumption, simplicity, and ease of integration into existing fossil fuel fired power plants. Selective membranes have been studied for the separation of gases for several hundred years. Many types of selective membranes exist, from the early use of pig bladders to modern inorganic membranes that require advanced synthesis techniques. Single-component membranes often exhibit sufficient performance for industrial separations in certain regards, but often at the expense of other properties. An approach to producing membranes with adequate properties for real-world applications relies on combining different materials, each with discrete advantages.
Mixed Matrix Membranes (MMMs) are systems comprised of at least two distinct components designed to complement and enhance the desired properties. MMMs usually consist of a major polymeric component that provides a suitable mechanical matrix and at least one additive component, usually an inorganic material, with high gas selectivity. Typically, MMMs often see significant enhancements in selectivity and/or permeability over single-component membranes. However, complex interactions between the components of MMMs often result in lower than expected enhancements or a reduction in other desirable properties. Many of the unexpected deficiencies in MMM properties are attributed to poor compatibility between the mixture components. Boundaries with defects, non-selective voids, plastic hardening and low gas transport properties often arise between mismatched MMM components that lack strong interfacial attractions.
Past approaches to overcome those deficiencies have focused on surface modification of inorganic additives to enhance attraction to the polymeric matrix. Those attempts have yielded some successes; however, unpredictability in interfacial properties still arises. This study will explore an approach that seeks to reduce the heterogenous nature of the interface between the components of the membrane, relying on porous molecular additives in contrast to traditional bulk additives. This approach results in more homogenous MMMs with additives being uniformly dispersed and incorporated into the polymeric matrix. Modification to the external functional groups of porous molecules allows for extensive control over the degree of intercalation of additives between polymer chains. Combining this approach with rigid network polymers that resist aging and exhibit intrinsic nanopores suited for the incorporation of porous molecular additives, a series of membranes with tuned interfacial properties for CO2 separation will be produced. The research is being conducted under the direction of Dr. Hongcai (Joe) Zhou.